To begin, it should first be understood that carbon monoxide binds to hemoglobin with an affinity of approximately 250 times greater than that of oxygen. The reason for this greater affinity is due to different enthalpic energy barriers that are encountered when either oxygen or carbon monoxide moves from the surrounding blood plasma to become bound to the central iron atom within the “heme pocket”. These enthalpic energy barriers are also equally important when either oxygen or carbon monoxide moves from the bound state and back towards the surrounding blood plasma on its way out.
Described simply, there are several small “energy hills” that must be overcome during this movement. In the case for oxygen, the height of the hills, and the valleys between the hills are all at the same “level” without any change in their baseline elevation. The oxygen molecule can therefore cross over these barriers with relative ease. Additionally, when the oxygen molecule gets over the last energy barrier to bind with the iron atom, it ends up in a “valley” that is not that deep. Thus, in moving back towards the surrounding blood plasma, it is not too difficult for the oxygen molecule to get over that first barrier to find its way out.
However, in the case for carbon monoxide, the landscape of the energy hills and valleys is much different. In moving from the surrounding blood plasma towards the iron atom, the height of the hills, and of the valleys between the hills, gradually slope upward so that there is a progressive rise of their baseline elevation. So when the carbon monoxide molecule finally gets over the last energy barrier to bind with the iron atom inside the heme pocket, it drops into a much deeper energy well. This deeper valley makes it 250 times more difficult for the carbon monoxide molecule to get over that first energy barrier in moving back towards the surrounding blood plasma to find its way out.
With an understanding of the above, the carbon monoxide molecule can benefit from somewhat “of a lift” to get over that first barrier. The energy of a single photon of light can indeed provide that extra push.
This is the underlying principle upon which this therapy is based. It was first demonstrated scientifically over fifty years ago using an investigative technique known as flash photolysis.
Photochemical research at that time revealed that the bond between carbon monoxide and the iron atom centered in either hemoglobin as well as in myoglobin can be broken by visible light.
In the discussion that follows, it is helpful to refer to the hemoglobin absorption spectra which is shown below.
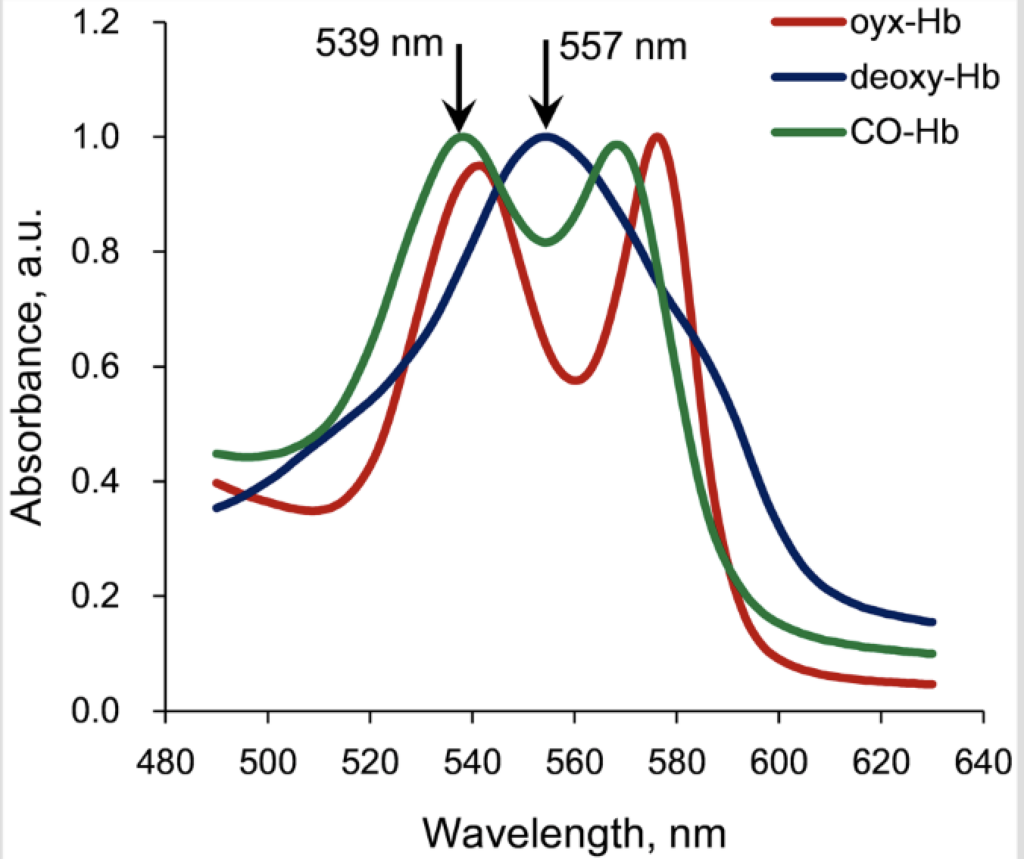
The photodissociation of carboxyhemoglobin occurs optimally at the two specific wavelengths of either 540 nm or 570 nm. Most importantly, the quantum efficiency for this photochemical reaction (using either 540 nm or 570 nm photons) is essentially 1.0. This means that for one incident photon that is absorbed, the carboxyhemoglobin bond is broken and one CO molecule is released.
Wavelengths other than these two would theoretically work, but not well, and the further one gets off the absorption peak, most of the light energy would be absorbed in the form of heat, which in itself, could also result in breaking the carboxyhemoglobin bond, but rather through an inefficient thermal effect.
Deoxyhemoglobin is hemoglobin without an attached oxygen molecule, so there is essentially not much to be gained by doing anything with deoxyhemoglobin, other than to get an oxygen molecule on-board, so that it becomes oxyhemoglobin.
With a close look at the absorption spectra, it can also be seen that oxyhemoglobin has an absorption peak very near 540 nm and that oxyhemoglobin has another absorption peak not too far from 570 nm. So an incident photon at 540 nm can also cause the photodissociation of oxyhemoglobin to become deoxyhemoglobin with the release of an oxygen molecule. However, the quantum efficiency of this photochemical reaction is quite low and is somewhere around 0.1. This means that for one incident photon that is absorbed, there is only about a 10% chance of breaking the bond and release the oxygen molecule. The absorption of the photon in this case mostly results in the transfer of heat energy.
It is also very important to know that each hemoglobin molecule is a tetramer, with two alpha subunits and two beta subunits. So each hemoglobin molecule can bind up to four oxygen molecules, which is why hemoglobin is such a good carrier of oxygen for the body.
Once any of the four binding sites is open and available, either oxygen or carbon monoxide can bind, and the binding by either of these two molecules is considered to be reversible.
However, formidable technical barriers existed for decades that prevented the translation of this knowledge from the physics laboratory to the bedside of severely poisoned patients.
It is to be noted that proof of principle of photodynamic therapy for carbon monoxide poisoning has already been established.
Photodissociation rates of carbon monoxide poisoned human blood resulting in a statistically significant reduction of carbon monoxide saturation over nonilluminated controls has been demonstrated in vitro under two different levels of luminance.
Direct transthoracic pulmonary phototherapy has also been shown to be an effective treatment for carbon monoxide poisoning in vivo in both a mouse model and a rat model. However, a 25 gram mouse or a 450 gram rat are quite small by human comparison.
Furthermore, in a study published in 2022, extracorporeal phototherapy of blood during veno-venous circulation demonstrated an increase in the rate of CO elimination in a pig model that used three-month old CO poisoned pigs with an average weight of 45 kg (99 pounds).